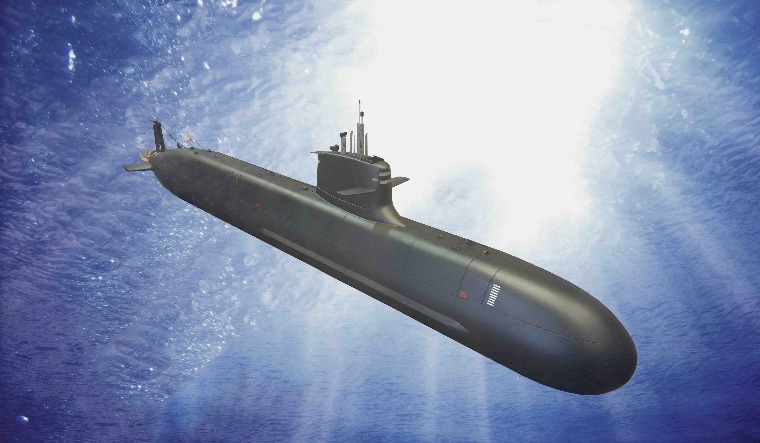
3
Carbon Dioxide
This chapter summarizes the relevant epidemiologic and toxicologic studies on carbon dioxide (CO2). Selected chemical and physical properties, toxicokinetic and mechanistic data, and inhalation exposure levels from the National Research Council (NRC) and other agencies are also presented. The subcommittee considered all of that information in its evaluation of the Navy’s current and proposed 1-hour (h), 24-h, and 90-day exposure guidance levels for CO2. The subcommittee’s recommendations for CO2 exposure levels are provided at the conclusion of this chapter along with a discussion of the adequacy of the data for defining those levels and the research needed to fill the remaining data gaps.
PHYSICAL AND CHEMICAL PROPERTIES
CO2 is a colorless, noncombustible gas with a faint acid taste (Budavari et al. 1989). It is reported to have either no odor (Budavari et al. 1989) or a faintly pungent odor (Ballou 1985). CO2 is heavier than air, and that contributes to the development of toxic exposure situations in enclosed spaces. Selected physical and chemical properties are listed in Table 3-1.
OCCURRENCE AND USE
CO2 has numerous applications. It is used in food freezing and chilling, beverage carbonation, chemical manufacture, fire prevention and extinction, metal working, and oil and gas recovery (Ballou 1985). It is produced on combustion of all carbonaceous fuels and is a product of animal metabolism. The annual average atmospheric concentration of CO2 is 372 parts per million (ppm) (Blasing and Jones 2003).
TABLE 3-1 Physical and Chemical Properties of Carbon Dioxidea
Synonyms and trade names |
Carbonic acid gas, carbonic anhydride, dry ice |
CAS registry number |
124-38-9 |
Molecular formula |
CO2 |
Molecular weight |
44.01 |
Boiling point |
— |
Melting point |
Sublimes at −78.48°C |
Flash point |
— |
Explosive limits |
— |
Specific gravity |
1.527 with respect to air |
Vapor pressure |
569.1 mmHg at −82°C |
Solubility |
Solubility in H2O at 20°C, 760 mmHg = 88 mL CO2/100 mL H2O; less soluble in alcohol and other neutral organic solvents |
Conversion factors |
1 ppm = 1.80 mg/m3; 1 mg/m3 = 0.56 ppm |
aData were taken from Budavari et al. (1989). Abbreviations: mg/m3, milligrams per cubic meter; mL, milliliters; mmHg, millimeters of mercury; ppm, parts per million; —, not available or not applicable. |
Submarine crew are reported to be the major source of CO2 on board submarines (Crawl 2003). Data collected on nine nuclear-powered ballistic missile submarines indicate an average CO2 concentration of 3,500 ppm with a range of 0-10,600 ppm, and data collected on 10 nuclear-powered attack submarines indicate an average CO2 concentration of 4,100 ppm with a range of 300-11,300 ppm (Hagar 2003).
SUMMARY OF TOXICITY
The information below was taken largely from a more comprehensive review, Spacecraft Maximum Allowable Concentrations for Selected Airborne Contaminants, Volume 2 (NRC 1996). The studies discussed represent those most relevant to submariners and the submarine environment.
CO2 is a simple asphyxiant and lethal asphyxiations have been reported at concentrations as low as 110,000 ppm (Hamilton and Hardy 1974). Loss of consciousness can occur within a minute of exposure at 300,000 ppm and within 5-10 minutes (min) of exposure at 100,000 ppm (HSDB 2004). The effects of concentrations of CO2 between 7,000 and 300,000 ppm in humans and animals are discussed below and include tremor, headaches, chest pain, respiratory and cardiovascular effects, and visual and other central nervous system (CNS) effects.
The respiratory, cardiovascular, and CNS effects of CO2 are related to the decreases in blood and tissue pH that result from exposures (Eckenhoff and Longnecker 1995; Yang et al. 1997; HSDB 2004). Changes in pH act directly and indirectly on those systems. The pH changes also trigger various compensatory mechanisms, including increased ventilation to reduce excess CO2 in the bloodstream, increased renal acid excretion to restore acid-base balance, and sympathetic nervous system stimulation to counteract the direct effects of pH changes on heart contractility and vasodilation (Eckenhoff and Longnecker 1995; HSDB 2004). The key effects for setting EEGL and CEGL values are tremor, headache, hyperventilation, visual impairment, and CNS impairment.
Effects in Humans
Accidental Exposures
In a case report of two men who lost consciousness in a wellhead chamber as a result of exposure to a “high concentration” of CO2 in the atmosphere, one man exhibited constricted visual fields, enlarged blind spots, photophobia, loss of convergence and accommodation, deficient dark adaptation, headaches, insomnia, and personality changes (Freedman and Sevel 1966). The other man died of asphyxia. In a similar incident, one overexposed man died after 11 months in a coma; he exhibited retinal atrophy and gliosis as well as loss of all ganglion cells (Sevel and Freedman 1967). In addition to that delayed fatality, three men died immediately from asphyxia. These studies are not relevant to establishing EEGL and CEGL values, but they are consistent with the findings that CO2 affects vision.
Experimental Studies
Experimental studies have shown that CO2 causes a variety of effects, ranging from nonspecific signs and symptoms, such as tremor, dyspnea, intercostal pain, and headache, to cardiovascular and CNS effects. Each of the effects mentioned is addressed in the following discussion.
Tremor was noted at a CO2 concentration of 60,000 ppm after several hours of exposure in a review that lacked documentation of specific methods (Schulte 1964). It was also noted in 10 of 12 subjects exposed at 7,000-14,000 ppm for 10-20 min (Sechzer et al. 1960). Tremor has not been reported at lower concentrations and, in fact, was specifically absent in many of the detailed neurobehavioral protocols discussed below.
Dyspnea is a commonly reported end point and can be induced by acute exposures to CO2 at >30,000 ppm (NRC 1996). Hyperventilation without dyspnea occurs at exposure concentrations as low as 10,000 ppm (NRC 1996). Dyspnea attributable to CO2 is aggravated by increasing the level of exertion. Studies at rest will be discussed, followed by discussion of those that included exercise protocols.
White et al. (1952) studied humans exposed to CO2 at 60,000 ppm for 16 min and reported that 19 of 24 subjects exhibited slight or moderate dyspnea and 5 of 24 exhibited severe dyspneic sensations. At 40,000-50,000 ppm for 17-32 min, 16 subjects reported dyspnea (Schneider and Truesdale 1922). In contrast, no dyspnea was reported in five subjects exposed at 32,000 ppm or at 25,000-28,000 ppm for several hours (Brown 1930).
In the most modern protocol to examine dyspnea, Menn et al. (1970) reported that eight subjects exposed to CO2 at 11,000 ppm exhibited no increase in dyspnea or intercostal pain during 30 min of maximal exercise. The same study reported that exposure to CO2 at 28,000 ppm during 30 min of maximal exercise produced increased dyspnea in three of eight subjects and intercostal pain in two of eight subjects, but subjects did not show increased dyspnea at one-half or two-thirds maximal exercise. Sinclair et al. (1971) reported that a 1-h exposure to CO2 at 28,000 ppm in four subjects caused no dyspnea or intercostal pain during steady strenuous exercise. Thus, the bulk of the data indicate a no-observed-adverse-effect level (NOAEL) for CO2 of about 28,000 ppm on the basis of the findings on dyspnea and intercostal pain.
Neither dyspnea nor intercostal pain occurred in four subjects exposed to CO2 at 28,000 ppm for 15-20 days and made to do 45 min of exercise twice daily at up to a heavy level, although the chronic portion of this protocol was not fully described (Sinclair et al. 1971). Similarly, there were no symptoms reported in six subjects exposed to CO2 at 20,000 ppm for 30 days or 29,000 ppm for 8 days and made to do 10 min of exercise twice a week at a workload of 150 watts (Guillerm and Radziszewski 1979; Radziszewski et al. 1988). Thus, 28,000 ppm is an appropriate chronic NOAEL for dyspnea and intercostal pain.
Headaches are commonly associated with increased CO2 concentrations in inspired air, but there is conflicting data on the concentrations reliably associated with that end point. There may also be an effect of exertion, because CO2 seems to cause more headaches at lower concentrations during exercise than it does during rest. In particular, Schneider and Truesdale (1922) reported that for 16 resting subjects exposed to CO2 at 10,000-80,000 ppm for 17-32 min, headaches developed only at concentrations that were ≥50,000 ppm; however, the headache could be intense. At 28,000 ppm for 1 h of strenuous steady-state exercise, occasional mild headaches were noted among four subjects (Sinclair et al. 1971). At 39,000 ppm for 30 min of exercise at two-thirds maximal exertion, Menn et al. (1970) found mild-to-moderate frontal headaches in six of eight subjects near the end of the exposure period. The headaches resolved after about an hour. At 28,000 ppm and 11,000 ppm for 30 min of exercise, no headaches were reported (Menn et al. 1970). Thus, there is inconsistent modern evidence for mild headaches resulting from CO2 exposures at 28,000 ppm during exercise. Some level of increased exertion among submarine crew might be likely during 1-24 h emergency episodes; however, headaches induced by CO2 are both mild and reversible and therefore were not used as a primary end point for setting the 1-h and 24-h EEGLs.
Subchronic CO2 exposures at 30,000 ppm or higher are known to produce headaches. Glatte et al. (1967) reported that CO2 at 30,000 ppm for 5 days led to mild to moderate throbbing frontal headaches on the first day in four of seven subjects. The headaches disappeared on day 3 and were not severe enough to interfere with normal activities, including 1 h of moderate exercise daily, although three of the four subjects with headaches requested analgesics. During 30-day exposures at 20,000 ppm, six subjects rarely developed headaches, and exposures at 29,000 ppm led to slight headaches (Radziszewski et al. 1988). Eight subjects, four exposed to CO2 at 28,000 ppm for 15-30 days and four exposed to CO2 at 39,000 ppm for 11 days, reported occasional mild headaches during exertion that disappeared after the first day of exposure (Sinclair et al. 1969, 1971). Thus, 20,000 ppm is an appropriate subchronic NOAEL for headaches.
CO2 is known to increase alveolar ventilation (hyperventilation), not as a toxic effect, but to maintain acid-base homeostasis. Concentrations as low as 10,000 ppm acutely increased ventilation by 32% in one study of 16 subjects exposed for 17-32 min (Schneider and Truesdale 1922). Increases in ventilation are thought to occur mainly through tidal volume increases, although increased respiratory rates have been reported in some studies. Chemoreceptors in brain and carotid bodies probably mediate the response, which sometimes persists after exposure is terminated to blow off CO2 and restore pH. The hyperventilation is also an adaptive response for preventing hypoxia in lower oxygen environments.
CO2 exposures at 50,000 or 75,000 ppm for 2 h resulted in decreased specific airway conductance, although exposures at 25,000 ppm did not. (Tashkin and Simmons 1972). Clinical indices of airway impairment were absent in this and other studies.
In summary, it takes an exposure concentration of at least 10,000 ppm to increase minute-volume after a plateau in the hyperventilatory response has been reached, usually after a few hours. It is not clear from the data whether the hyperventilatory response diminishes with time, although in a study at 10,000 ppm, it resolved completely after 8 days of a 44-day exposure (Pingree 1977). Data from Radziszewski et al. (1988) showed a 60% increase in minute-volume during a 2-h exposure at 20,000 ppm. The increase was reduced to 45% after 24 h. There is no indication in the literature that hyperventilation constitutes an adverse response.
Exposures to CO2 at concentrations much higher than those in ambient air lead to increased partial pressure of CO2 in alveoli and blood. That causes a lowering of blood pH, which is eventually buffered by blood proteins and bicarbonate. During a 1-h exposure at 28,000 ppm, rapid acidosis occurred after 45 min of mild to moderate exercise, but the acidosis did not impair function in this study, even with prolonged exposures of up to 20 days with 45 min of exercise twice daily (Sinclair et al. 1971). Guillerm and Radziszewski (1979) reported similar results in a study of a 30-day exposure at 20,000 ppm that included twice weekly 10-min exercise periods. Thus, acidosis does not seem to be an end point of concern for setting 1-h and 24-h standards.
CO2 exposures as low as 7,000 ppm can lower blood pH by up to 0.05 units, but even at high exposures, renal compensation seems to occur in healthy subjects. In a 30-day exposure to CO2 at 20,000 ppm, there was an average pH change of only 0.01 units (Guillerm and Radziszewski 1979). Compensation occurs over a variable period of time, but effects of lowered pH on clinical status or performance have not been reported either experimentally or operationally (Schaefer et al. 1964a).
Exposures to CO2 at 10,000-20,000 ppm for 17-32 min were reported to cause slight increases in systolic and diastolic blood pressure (Schneider and Truesdale 1922). Exposures at 50,000 ppm or 70,000 ppm for 15-30 min caused increases in blood pressure but no changes in cardiac output (Kety and Schmidt 1948). Grollman (1930) reported increases in cardiac output and heart rate during 4-25 min exposures at 75,000 ppm.
Electrocardiograph changes resulting from CO2 exposures have received much attention. A number of changes, including atrial tachycardia and increased QT intervals, were found during exposures at about 300,000 ppm (in 70% oxygen) (MacDonald and Simonson 1953; McArdle 1959). In the more moderate range of 70,000-140,000 ppm, various clinically unimportant rhythm changes, such as premature nodal contractions and rare premature ventricular contractions, have been reported (Sechzer et al. 1960). Glatte et al. (1967) found no electrocardiograph problems in individuals exposed to CO2 at 30,000 ppm for 5 days, during which they exercised for 1 h daily. Sinclair et al. (1971) found no increases in premature ventricular contractions at 28,000 ppm for 15-20 days during which subjects were made to engage in moderate and heavy exercise, although this chronic protocol was not adequately described. On the basis of data from Glatte et al. (1967) and Sinclair et al. (1971), subchronic exposures at 30,000-40,000 ppm appear to be free of significant arrhythmia effects. At lower concentrations, chronic exposures resulted in only minor electrocardiograph changes without any rhythm disturbances (Radziszewski et al. 1988). Thus, cardiovascular end points are not of primary concern for setting the EEGL or CEGL values for CO2.
Guillerm and Radziszewski (1979) reported a 10% reduction in hematocrit and a 9% reduction in red blood cell count in subjects exposed to CO2 at 20,000 ppm for 30 days. These changes were not observed by the same investigators at 40,000 ppm, which makes them of dubious significance. The investigators attributed them to prolonged confinement rather than to CO2 exposure. Wilson and Schaefer (1979) found that on Polaris submarine patrols that had measured CO2 concentrations between 7,000 and 12,000 ppm and carbon monoxide concentrations of 15-20 ppm, the hematology of smokers differed from that of nonsmokers. In the nine smokers examined, red blood cell count increased by a statistically significant 12% on day 6, but returned to near baseline by day 52. However, in 11 nonsmokers, there was no statistically significant change in red blood cell count on any of the 3 examination days. Although the findings might suggest a differential response in smokers exposed to CO2, they cannot be used to set CO2 standards.
While on active submarine patrol for 57 days, 7 out of 15 crewmen exposed to CO2 at 8,000-12,000 ppm developed decreased plasma calcium and increased erythrocyte calcium (Messier et al. 1976). There were no changes in parathyroid hormone or calcitonin (Messier et al. 1976). Some observational data from submarine patrols documented increased urinary calculi in crewmen when CO2 was present at >10,000 ppm most of the time, instead of at <10,000 ppm (Tansey et al. 1979). There are several physiologic reasons why that is not thought to be causal; in particular, the incidence rate of urinary calculi observed in submariners does not seem to exceed the general population rate. Exposure to CO2 at 50,000 ppm for 30 min led to increased renal blood flow, glomerular filtration rate, and renal venous pressure, as well as increased renal vascular resistance (Yonezawa 1968). These physiologic changes related to renal compensation for CO2-induced acidosis are considered to be innocuous. Thus, electrolyte, bone, and kidney effects are not appropriate end points for developing exposure standards.
It is well established that CO2 acutely impairs vision and hearing at concentrations exceeding about 50,000 ppm (Yang et al. 1997). Exposures to CO2 at 61,000-63,000 ppm for 6 min led to 3-8% increases in the hearing threshold for six subjects (Gellhorn and Spiesman 1935). The same authors noted slight impairment after 5-22 min of exposure at 30,000-40,000 ppm and identified a NOAEL of 25,000 ppm (Gellhorn and Spiesman 1934, 1935).
Sun et al. (1996) studied the effects of CO2 exposures at 25,000 ppm on stereoacuity (depth) perception in three adult subjects—two males and one female—using a two-alternative forced-choice procedure. The exposure duration was not stated, but the subcommittee estimated the duration to be about 1 h on the basis of the 30-min acclimatization period followed by the testing session. The psychometric functions curves of all three subjects shifted to the right, but returned to baseline after 2 h of breathing fresh air. Stereoacuity values, the reciprocal of stereoscopic thresholds, were statistically significantly reduced in all three subjects. In a second study, Yang et al. (1997) investigated the effects of CO2 exposures at 25,000 ppm on perception of coherent motion in three subjects with normal or corrected-to-normal vision using a two-interval forced-choice psychophysical procedure. The exposure duration appears to have been 1 h. Motion detection thresholds were statistically significantly different during exposure in all three subjects, but the subjects showed complete recovery in fresh air. Although the number of subjects was small, these two studies are considered to be methodologically rigorous and involve more sensitive end points than those discussed previously. These studies suggest an acute lowest-observed-adverse-effect level (LOAEL) for visual effects of 25,000 ppm.
Exposures at 50,000-67,500 ppm in 19.2% oxygen for 37 h caused decreased hand-arm steadiness but caused no changes in computing, translating, number checking, or discrimination of pitch or loudness in four subjects (Consolazio et al. 1947). Among fighter pilots, exposures to CO2 at 50,000 ppm degraded performance in multiple aspects of simulated landing (Wamsley et al. 1969), clearly indicating that CO2 at 50,000 ppm impairs neurobehavioral performance.
Brown (1930) studied five subjects exposed to CO2 concentrations that ranged from 41,000 ppm to 53,000 ppm for 8 h. Results showed a statistically significant 24% decrease in number cancellation in a number cancellation test. The same exposures did not affect performance on the Army Alpha intelligence and arithmetic tests, attention, or muscular coordination, leaving open the possibility that the 24% decrease in number cancellations from nonspecific responses was the result of confinement in the chamber (Brown 1930). The Brown study did not include any control subjects.
A number of studies suggest that CO2 exposures in the range of 15,000-40,000 ppm do not impair neurobehavioral performance. Schaefer (1961) reported that 23 crewmen exposed to CO2 at 15,000 ppm for 42 days in a submarine showed no psychomotor testing effects but showed moderate increases in anxiety, apathy, uncooperativeness, desire to leave, and sexual desire. In a 5-day exposure of seven subjects at a CO2 concentration of 30,000 ppm, Glatte et al. (1967) reported no effects on hand steadiness, vigilance, auditory monitoring, memory, or arithmetic and problem solving performance. Storm and Giannetta (1974) studied the effects of 2 weeks of exposure to CO2 at 40,000 ppm on psychomotor performance in a 6-week protocol that included a 2-week pre-exposure baseline period and 2 weeks of recovery. Twenty-four volunteers, ages 18-23, were selected for their motivation and their excellent health. Two experimental groups and two control groups of six subjects each were formed. One exposure group and one control group were on bedrest to simulate weightlessness; the others were active. The primary outcome measure was a tracking task with a joy stick and rudder. Acute effects were not measured. All measurements and practice were done in the pre-exposure and recovery periods. An additional outcome measure, the repetitive psychometric measures (RPM) test, was used. It is a six-part pencil and paper test that measures complex cognitive tasks, such as aiming, flexibility of closure, perceptual speed, visualization, number facility, and speed of closure. Using 20 versions of the subtests minimized practice effects, and the longitudinal design sought to avoid memorization.
CO2 exposure did not affect performance on the tracking task or any of the six RPM subtests (Storm and Giannetta 1974). There was a learning effect for the tracking task during both pre-exposure and recovery, but the authors still thought it was appropriate to conclude the absence of a performance impairment. The authors considered it especially likely because previous papers had suggested that impairment is easier to detect during skill reacquisition, which occurred following the 2 weeks of exposure without practice, rather than at an asymptotic skill level (Storm and Giannetta 1974). Thus, CO2 at 40,000 ppm for 2 weeks did not affect performance on multiple tests of cognitive function in physically fit young airmen, a population probably not unlike submariners.
Based on the work of Storm and Giannetta (1974) and Glatte et al. (1967), a NOAEL of 30,000 ppm for general CNS effects could be proposed. However, the subcommittee considers the subtler, if less relevant, visual effects reported by Sun et al. (1996) and Yang et al. (1997) at 25,000 ppm to be a minimal LOAEL.
Occupational and Epidemiologic Studies
In a review by Schulte (1964), exposure to CO2 at 30,000 ppm caused dyspnea at rest. Exposures at 20,000-30,000 ppm for several hours caused headaches on mild exertion, and the headaches at 30,000 ppm were more severe than those at 20,000 ppm (Schulte 1964). CNS depression developed after several hours of exposure at 50,000 ppm (Schulte 1964). A CO2 exposure at 30,000 ppm for 8 days in a working submarine crew (unknown number of subjects) led to clinical observations of euphoria and troubled sleep on day 1 and poor attention, erratic behavior, confusion, and motor skill impairment on days 2-8 (Schaefer 1949a,b). Schaefer (1958, 1959, 1963) reported that some crewmen of a German submarine exposed to CO2 at 30,000-35,000 ppm in 15-17% oxygen during a 2-month underwater patrol in World War II suffered from impaired attentiveness. The authors did not consider confounding contaminants or the low oxygen concentrations aboard the submarines, and thus it is problematic to rely on these uncontrolled studies.
In the only nonmilitary study, brewery workers exposed during an 8-h work shift to a time-weighted average concentration of CO2 at 11,000 ppm and to excursions at up to 80,000 ppm for 3 min had blood bicarbonate levels that were no different from controls, consistent with the relatively mild impact of chronic CO2 exposures on electrolytes and acid-base balance (NIOSH 1976).
Effects in Animals
Changes in lung histopathology, changes in liver and heart, and gastrointestinal bleeding associated with CO2 exposures were reported in animal studies; however, the quality and relevance of those data is questionable. Those effects are discussed below for the purpose of completeness.
Schaefer et al. (1964b) found lung effects in guinea pigs exposed to CO2 at 150,000 ppm for durations ranging from 1 to 24 h. The effects observed included subpleural atelectasis, increased lamellar bodies in alveolar lining cells, congestion, edema, hemorrhage, increases in phagocytic pneumocytes, increased lung-to-body weight ratios, increased surface tension of lung extracts, and increases in hyaline membranes. However, in a 14-day experiment at 150,000 ppm, no changes in lung-to-body weight ratios or surface tension of lung extracts, and no subpleural atelectasis, edema, hemorrhage, or abnormal lamellar bodies in alveolar lining cells were observed (Schaefer et al. 1964b). At 30,000 ppm for 2 days, subpleural atelectasis and edema, but no hyaline membranes, were found in guinea pigs, and at 15,000 ppm for 6 months, only subpleural atelectasis was found (Niemoller and Schaefer 1962). In addition to having somewhat mixed findings and extremely high exposures, the studies had inadequate numbers of control animals (NRC 1996) and were not further considered.
Douglas et al. (1979) reported the proliferation of type II pneumocytes during a 4-week exposure to CO2 at as little as 10,000 ppm. Gas exchange was not impaired, and the effect was considered to be a metabolic adaptation of the lungs to CO2 (Douglas et al. 1979). The subcommittee thought that these changes were functionally insignificant, especially given that they are inconsistent with the body of literature on human experiences. Thus, these studies were not used for setting the CEGL.
In an uncontrolled study by Meessen (1948), rabbits exposed at 45,000 ppm in 21% oxygen for 13 days were found to have scattered necrotic areas throughout the liver lobules. Pepelko (1970) did not observe histologic effects in liver, lungs, kidneys, spleen, thyroid, adrenals, or heart in rats exposed to CO2 at 80,000 ppm for 32 days. Schaefer et al. (1971) found a decrease in glycogen granules and an increase in fat granules in the livers of guinea pigs exposed to CO2 at 30,000 ppm for 7 days. These observed changes were thought to reflect functional changes in liver metabolism but were not considered liver damage.
Increased fat deposition was seen in the myocardia of guinea pigs exposed to CO2 at 150,000 ppm for 7 days (Schaefer et al. 1971). Gastrointestinal bleeding was observed in dogs exposed to CO2 at 150,000 ppm for 3 h and in guinea pigs exposed at 150,000 ppm for 24 h (DeBellis et al. 1968; Schaefer et al. 1971). These results are not considered to be relevant for setting exposure guidelines because of the extremely high exposure concentrations used.
Reproductive Toxicity in Males
Exposures to CO2 at 25,000 ppm, 50,000 ppm, and 100,000 ppm for 4-8 h in rats produced a concentration-dependent disappearance of mature spermatids attributed to sloughing of mature spermatids and Sertoli cells in the seminiferous tubules (Vandemark et al. 1972). There were no effects after a 1- or 2-h exposure, and there was complete structural recovery within 36 h, so this end point was not used to set the EEGL and CEGL values. Mukherjee and Singh (1967) exposed mice to CO2 at 360,000 ppm in 13.4% oxygen alternating 2 h of exposure with 30 min of fresh air for 6 h in one experiment and exposing the mice for 4 h per day for 6 days in another experiment. They found spermatozoa with smaller heads and midpieces in the vas deferens in the short-term experiment and reduced fertility in the 6-day experiment. The very high CO2 concentrations and low oxygen concentrations make this study inappropriate for setting EEGL and CEGL values.
Immunotoxicity
No relevant information was found regarding the potential immunotoxicity of CO2.
Genotoxicity
No relevant information was found regarding the potential genotoxicity of CO2.
Carcinogenicity
No relevant information was found regarding the potential carcinogenicity of CO2.
TOXICOKINETIC AND MECHANISTIC CONSIDERATIONS
CO2 freely penetrates cell membranes and diffuses from lungs to blood at a rate 20 times faster than oxygen because CO2 has much greater solubility (West 1979). CO2 undergoes catalysis by the enzyme carbonic anhydrase in red blood cells to form carbonic acid, which is then ionized to bicarbonate (Baggott 1982). Thus, 90% of the CO2 in the body is carried in blood as bicarbonate ion. Of the remaining CO2, 5% forms carbamino compounds in the reaction of CO2 with uncharged amino groups on hemoglobin and 5% is dissolved in serum and cytoplasm (Baggott 1982). At rest, CO2 is exhaled at about 220 milliliters per minute (mL/min), increasing to 1,650 mL/min during moderate exercise (Cotes 1979).
CO2-induced pH changes directly dilate blood vessels and decrease cardiac contractility. CO2 also stimulates the sympathetic nervous system, increasing blood concentrations of epinephrine, norepinephrine, and angiotensin to counteract the direct cardiovascular effects (Staszewska-Barczak and Dusting 1981). The sympathetic response does not fully compensate for the direct vasodilation (Eckenhoff and Longnecker 1995).
The subtle visual effects of CO2 exposure might be related to the changes in neurotransmitter levels and activity caused by CO2-related pH changes (Yang et al. 1997). Excess CO2 in blood depresses the excitability of the cerebral cortex (Eckenhoff and Longnecker 1995). At CO2 concentrations of 250,000 ppm and greater, subcortical areas that have cortical projections are activated, overcoming the depressant effect and sometimes resulting in convulsions (Eckenhoff and Longnecker 1995). Concentrations at 500,000 ppm or greater produce marked cortical and subcortical depression similar to the action of anesthetics (Eckenhoff and Longnecker 1995).
INHALATION EXPOSURE LEVELS FROM THE NRC AND OTHER ORGANIZATIONS
A number of organizations have established or proposed acceptable inhalation exposure limits or guidelines for CO2. Selected values are summarized in Table 3-2.
SUBCOMMITTEE RECOMMENDATIONS
The subcommittee’s recommendations for EEGL and CEGL values for CO2 are summarized in Table 3-3. The current and proposed U.S. Navy values are provided for comparison.
Organization |
Type of Level |
Exposure Level (ppm) |
Reference |
Occupational |
|
|
|
ACGIH |
TLV-Ceiling |
5,000 |
ACGIH 2002 |
|
TLV-STEL |
30,000 |
|
NIOSH |
REL-TWA |
5,000 |
NIOSH 2004 |
|
REL-STEL |
30,000 |
|
OSHA |
PEL-TWA |
5,000 |
29 CFR 1910.1000 |
Spacecraft |
|
|
|
NASA |
SMAC |
|
NRC 1996 |
|
1 h |
13,000 |
|
|
24 h |
13,000 |
|
|
30 days |
7,000 |
|
|
180 days |
7,000 |
|
aThe comparability of EEGLs and CEGLs with occupational and public health standards or guidance levels is discussed in Chapter 1, section “Comparison to Other Regulatory Standards or Guidance Levels.” Abbreviations: ACGIH, American Conference of Governmental Industrial Hygienists; h, hour; NASA, National Aeronautics and Space Administration; NIOSH, National Institute for Occupational Safety and Health; OSHA, Occupational Safety and Health Administration; PEL, permissible exposure limit; ppm, parts per million; REL, recommended exposure limit; SMAC, spacecraft maximum allowable concentration; STEL, short-term exposure limit; TLV, Threshold Limit Value; TWA, time-weighted average. |
1-Hour EEGL
Visual impairment and CNS effects are the most appropriate end points for determining the 1-h EEGL. Two studies, Glatte et al. (1967) and Storm and Giannetta (1974), used the repetitive psychometric measures (RPM) tests on multiple subjects in experiments ranging from 5 to 14 days duration and found subchronic NOAELs for visual effects and tremors of 30,000 ppm and 40,000 ppm, respectively. Studies at exposure concentrations of 28,000-29,000 ppm for 30 min to 1 h and up to 8 days reported no CNS effects (Menn et al. 1970; Sinclair et al. 1971; Radziszewski 1988). However, Sun et al. (1996) and Yang et al. (1997) found asymptomatic decrements in stereoacuity and motion perception in a total of six subjects exposed at 25,000 ppm for durations of about 1 h. The effects were fairly subtle, the number of subjects was small, the effects were rapidly reversible, and the toxicologic and operational significance is questionable.
Exposure Level |
U.S. Navy Values (ppm) |
NRC Recommended Values (ppm) |
||
Current |
Proposed |
|||
EEGL |
|
|
|
|
|
1 h |
40,000 |
30,000 |
25,000 |
|
24 h |
40,000 |
15,000 |
25,000 |
CEGL |
|
|
|
|
|
90 days |
5,000 |
7,000 |
8,000 |
Abbreviations: CEGL, continuous exposure guidance level; EEGL, emergency exposure guidance level; h, hour; NRC, National Research Council; ppm, parts per million. |
Storm and Giannetta (1974) could be used to establish 40,000 ppm as a NOAEL for clinically apparent CNS effects (visual disturbances, tremor, and neurobehavioral impairment). The 40,000-ppm exposure was well tolerated, and the study included many tests relying on visual function. However, the Sun et al. (1996) and Yang et al. (1997) studies reported a LOAEL at 25,000 ppm on the basis of subtle and asymptomatic errors of visual tracking and depth perception. Because the exposure guidance must be protective in the low-oxygen atmospheres of submarines, the minimal LOAEL of 25,000 ppm is recommended for the 1-h EEGL.
24-Hour EEGL
For the 24-h EEGL, it is noted that the exposure studies discussed above for determining the 1-h EEGL, including the Storm and Giannetta (1974) study, included exposure durations of up to 2 weeks and reported no significant adverse effects at concentrations up to 40,000 ppm. For that reason, no reduction in the 1-h EEGL is warranted for extrapolation to 24 h, and 25,000 ppm also is recommended for the 24-h EEGL.
90-Day CEGL
For a 90-day exposure, the Sun et al. (1996) and Yang et al. (1997) visual function findings are of greater concern, especially given that there was no available 90-day study of neurobehavioral effects of CO2 exposures. Thus, using the Sun et al. (1996) and Yang et al. (1997) 25,000-ppm LOAEL as the basis for the CEGL and adjusting it with an uncertainty factor of 3 for limited data on the effects of longer-term exposure yields a 90-day CEGL of 8,000 ppm. The subcommittee does not expect that a 8,000-ppm level will cause long-term neurobehavioral changes given the work of Storm and Giannetta (1974), which included exposures at up to 40,000 ppm for durations of up to two weeks and reported no significant adverse effects on several psychometric tests. The subcommittee also considers a 8,000-ppm level to be protective against other end points, such as headache and metabolic and acid-base changes, that have been studied.
The subcommittee considered the potential secondary effects of hyperventilation associated with CO2 inhalation. The research cited above offers little information directly pertinent to that issue. There is no evidence that dyspnea and intercostal pain are time dependent. Although the NOAEL for 30 days is 28,000 ppm, hyperventilation and associated symptoms occur at lower levels. Under most conditions, CO2-induced hyperventilation is not harmful, and it is an adaptive response when oxygen is displaced by CO2 at an abnormally high level. The secondary toxic effects of CO2-induced hyperventilation that should be considered include (1) discomfort associated with extreme hyperventilation, (2) impairment of ability to exercise or to take on an extreme workload, and (3) increased inhalation of toxicants.
Sinclair et al. (1969) reported that four subjects exposed to CO2 at 28,000 ppm for 30 days and another four subjects exposed to CO2 at 39,000 ppm for 11 days tolerated hyperventilation “without apparent difficulty.” Radziszewski et al. (1988) and Guillerm and Radziszewski (1979) found no symptoms in six subjects exposed to CO2 at 20,000 ppm for 30 days, although minute-volumes increased about 40% during the first several days of the study. Sinclair et al. (1971) found that four male subjects could perform 45 min of light, moderate, and heavy steady-state exercise twice daily during a 15-20 day exposure to CO2 at 28,000 ppm.
The possibility of increased inhalation of other toxicants as a result of CO2-induced hyperventilation must be addressed. In Pingree (1977), minute-volumes rose 30% by day 4 and returned to baseline by day 8 of a 44-day study in 15 subjects exposed to CO2 at 10,000 ppm. A 30% increase in ventilation is modest in comparison with increases normally associated with exercise (for example, about 180% increase with light exercise) (Sinclair et al. 1971). Because CO2-induced changes disappear within a few days, no significant increases in toxicant exposures are expected (NRC 1996).
The neurobehavioral studies on which the 1-h EEGL is based were conducted no more recently than the 1970s except for the small studies of Sun et al. (1996) and Yang et al. (1997). More sensitive tests and tests specifically designed to evaluate the skills required for high technology equipment use and onboard decision making might be available. It is important to validate the Sun et al. (1996) and Yang et al. (1997) findings, because they suggest significantly lower acceptable concentrations than do previous studies. Also, subchronic studies should be repeated to evaluate more sensitive end points and should include complete lung function tests with diffusing capacity as well as neurobehavioral tests.
REFERENCES
ACGIH (American Conference of Governmental Industrial Hygienists). 2002. Threshold Limit Values (TLVs) for Chemical Substances and Physical Agents and Biological Exposure Indices (BEIs) for 2002. Cincinnati, OH: American Conference of Governmental Industrial Hygienists.
Baggott, J. 1982. Gas transport and pH regulation. Pp. 1098-1101, 1114, 1120-1123 in Textbook of Biochemistry with Clinical Correlations, T.M. Devlin, ed. New York: John Wiley and Sons (as cited in NRC 1996).
Ballou, W.R. 1985. Carbon dioxide. Pp. 212-213 in Kirk-Othmer Concise Encyclopedia of Chemical Technology, M. Grayson, D. Eckroth, E. Graber, A. Klingsberg, and P.M. Siegel, eds. New York: John Wiley and Sons.
Blasing, T.J., and S. Jones. 2003. Current Greenhouse Gas Concentrations. CDIAC, Carbon Dioxide Information Analysis Center [Online]. Available: http://cdiac.esd.ornl.gov/pns/current_ghg.html [accessed March 22, 2004].
Brown, E.W. 1930. The physiological effects of high concentrations of carbon dioxide. U.S. Naval Med. Bull. 28:721-934 (as cited in NRC 1996).
Budavari, S., M.J. O’Neil, A. Smith, and P.E. Heckelman, eds. 1989. Carbon Dioxide. P. 274 in The Merck Index: An Encyclopedia of Chemicals, Drugs, and Biologicals, 11th Ed. Rahway, NJ: Merck and Co.
Crawl, J.R. 2003. Review/Updating of Limits for Submarine Air Contaminants. Presentation at the First Meeting on Emergency and Continuous Exposure Guidance Levels for Selected Submarine Contaminants, January 23, 2003, Washington, DC.
Consolazio, W.B., M.B. Fisher, N. Pace, L.J. Pecora, and A.R. Behnke. 1947. Effects on man of high concentration of carbon dioxide in relation to various oxygen pressures during exposures as long as 72 hours. Am. J. Physiol. 151:479-503 (as cited in NRC 1996).
Cotes, J.E. 1979. Lung function: Assessment and Application in Medicine, 4th Ed. Oxford, U.K.: Blackwell Science Publications (as cited in NRC 1996).
Douglas, W.H.J., K.E. Schaefer, A.A. Messier, and S.M. Pasquale. 1979. Proliferation of pneumocyte II cells in prolonged exposure to 1% CO2. Undersea Biomed. Res. (Submarine Suppl.):S135-S142 (as cited in NRC 1996).
Eckenhoff, R.G., and D.E. Longnecker. 1995. The therapeutic gases. Effects of carbon dioxide. Pp. 355-356 in Goodman and Gilman’s The Pharmacological Basis of Therapeutics, 9th Ed., J.G. Hardman, L.E. Limbird, P.B. Molinoff, R.W. Ruddon, and A.G. Gilman, eds. New York: McGraw Hill.
Freedman, A., and D. Sevel. 1966. The cerebro-ocular effects of carbon dioxide poisoning. Arch. Opthalmol. 76(1):59-65.
Glatte, Jr., H.A., G.J. Motsay, and B.E. Welch. 1967. Carbon Dioxide Tolerance Studies. Rep. No. SAM-TR-67-77. Aerospace Medical Division, USAF School of Aerospace Medicine, Brooks Air Force Base, San Antonio, TX (as cited in NRC 1996).
Gellhorn, E., and I. Spiesman. 1934. Influence of variations of O2 and CO2 tension in inspired air upon hearing. Proc. Soc. Exp. Biol. Med. 32:46-47.
Gellhorn, E., and I. Spiesman. 1935. Influence of hypercapnea and of variations of O2– and CO2-tension in inspired air upon hearing. Am. J. Physiol. 112:519-528 (as cited in NRC 1996).
Grollman, A. 1930. Physiological variations in the cardiac output of man. IX. The effect of breathing carbon dioxide, and of voluntary forced ventilation on the cardiac output of man. Am. J. Physiol. 94:287-299 (as cited in NRC 1996).
Guillerm, R., and E. Radziszewski. 1979. Effects on man of 30-day exposure to a PI CO2 of 14 torr (2%): Application to exposure limits. Undersea Biomed. Res. (Submarine Suppl.): S91-S114.
Hagar, R. 2003. Submarine Atmosphere Control and Monitoring Brief for the COT Committee. Presentation at the First Meeting on Emergency and Continuous Exposure Guidance Levels for Selected Submarine Contaminants, January 23, 2003, Washington, DC.
Hamilton, A., and H.L. Hardy. 1974. Carbon dioxide. Pp. 236-237 in Industrial Toxicology, 3rd Ed. Acton, MA: Publishing Sciences Group, Inc. (as cited in HSDB 2004).
HSDB (Hazardous Substances Data Bank). 2004. Carbon dioxide. TOXNET, Specialized Information Services, U.S. National Library of Medicine, Bethesda, MD. [Online]. Available: http://toxnet.nlm.nih.gov/cgi-bin/sis/htmlgen?HSDB [accessed March 1, 2004].
Kety, S.S., and C.F. Schmidt. 1948. The effects of altered arterial tensions of carbon dioxide and oxygen on cerebral flood flow and cerebral oxygen consumption of normal young men. J. Clin. Invest. 27:484-492 (as cited in NRC 1996).
MacDonald, F.M., and E. Simonson. 1953. Human electrocardiogram during and after inhalation of thirty percent carbon dioxide. J. Appl. Physiol. 6(5):304-310 (as cited in NRC 1996).
McArdle, L. 1959. Electrocardiographic studies during the inhalaion of 30 percent carbon dioxide in man. Br. J. Anaesth. 31(4):142-151 (as cited in NRC 1996).
Meessen, H. 1948. Chronic carbon dioxide poisoning experimental studies. Arch. Pathol. 45:36-40 (as cited in NRC 1996).
Menn, S.J., R.D. Sinclair, and B.E. Welch. 1970. Effect of inspired pCO2 up to 30 mm Hg on response of normal man to exercise. J. Appl. Physiol. 28(5):663-671 (as cited in NRC 1996).
Messier, A.A., E. Heyder, W.R. Braithwaite, C. McCluggage, A. Peck, and K.E. Schaefer. 1976. Calcium, magnesium, and phosphorus metabolism, and parathyroid-calcitonin function during prolonged exposure to elevated CO2 concentrations on submarines. Undersea Biomed. Res. 6(Suppl.):S57-S70 (as cited in NRC 1996).
Mukherjee, D.P., and S.P. Singh. 1967. Effect of increased carbon dioxide in inspired air on the morphology of spermatozoa and fertility of mice. J. Reprod. Fertil. 13(1):165-167 (as cited in NRC 1996).
Niemoller, H., and K.E. Schaefer. 1962. Development of hyaline membranes and atelectasis in experimental chronic respiratory acidosis. Proc. Soc. Exp. Biol. Med. 110:804-808 (as cited in NRC 1996).
NIOSH (National Institute for Occupational Safety and Health). 1976. Occupational Exposure to Carbon Dioxide, Criteria for a Recommended Standard. DHEW (NIOSH) Publication No. 76-194. Public Health Service, Center for Disease Control, National Institute for Occupational Safety and Health, U.S. Department of Health, Education, and Welfare, Cincinnati, OH.
NIOSH (National Institute for Occupational Safety and Health). 2004. NIOSH Pocket Guide to Chemical Hazards. DHHS (NIOSH) Publication No. 2004-103. National Institute for Occupational Safety and Health, Centers for Disease Control and Prevention, U.S. Department of Health and Human Services, Cincinnati, OH.
NRC (National Research Council). 1996. Carbon dioxide. Pp. 105-189 in Spacecraft Maximum Allowable Concentrations for Selected Airborne Contaminants, Vol. 2. Washington, DC: National Academy Press.
Pepelko, W.E. 1970. Effects of hypoxia and hypercapnia, singly and combined, on growing rats. J. Appl. Physiol. 28:646-651 (as cited in NRC 1996).
Pingree, B.J.W. 1977. Acid base and respiratory changes after prolonged exposure to 1% carbon dioxide. Clin. Sci. Mol. Med. 52(1):67-74 (as cited in NRC 1996).
Radziszewski, E., L. Giacomoni, and R. Guillerm. 1988. Effets physiologiques chez l’homme du confinement du longue duree en atmosphere enrichie en dioxyde de carbone. Pp. 19-23 in Proceedings of the Colloquium on Space and Sea [in French]. European Space Agency, Brussels, Belgium (as cited in NRC 1996).
Schaefer, K.E. 1949a. Influence exerted on the psyche and the excitatory processes in the peripheral nervous system under long-term effects of 3% CO2 [in German]. Pfluegers Arch. Gesamte. Physiol. Menschen Tiere 251:716-725 (as cited in NRC 1996).
Schaefer, K.E. 1958. Effects of Carbon Dioxide as Related to Submarines and Diving Physiology. Memo. Rep. No. 58-11. Naval Medical Research Laboratory, New London, CT (as cited in NRC 1996).
Schaefer, K.E. 1959. Experiences with submarine atmospheres. J. Aviat. Med. 30(5):350-359.
Schaefer, K.E. 1961. A concept of triple tolerance limits based on chronic carbon dioxide toxicity studies. Aeromed. Acta. 32:197-204.
Schaefer, K.E. 1963. The effects of CO2 and electrolyte shifts on the central nervous system. Pp. 101-123 in Selective Vulnerability of the Brain in Hypoxia, J.P. Schade, ed. Oxford, U.K.: Blackwell Scientific Publications (as cited in NRC 1996).
Schaefer, K.E., G. Nichols, Jr., and C.R. Carey. 1964a. Acid-base balance and blood and urine electrolytes of man during acclimatization to CO2. J. Appl. Physiol. 19:48-58.
Schaefer, K.E., M.E. Avery, and K. Bensch. 1964b. Time course of changes in surface tension and morphology of alveolar epithelial cells in CO2-induced hyaline membrane disease. J. Clin. Invest. 43(11):2080-2093 (as cited in NRC 1996).
Schaefer, K.E., H. Niemoller, A. Messier, E. Heyder, and J. Spencer. 1971. Chronic CO2 Toxicity: Species Difference in Physiological and Histopathological Effects. Rep. No. 656. Naval Submarine Medical Research Laboratory, Groton, CT (as cited in NRC 1996).
Schneider, E.C., and D. Truesdale. 1922. The effects on the circulation and respiration of a increase in carbon dioxide content of the blood in man. Am. J. Physiol. 63:155-175 (as cited in NRC 1996).
Schulte, J.H. 1964. Sealed environments in relation to health and disease. Arch. Environ. Health 85:438-452.
Sechzer, J.H., L.D. Egbert, H.W. Linde, D.Y. Cooper, R.D. Dripps, and H.L Price. 1960. Effect of CO2 inhalation on arterial pressure, ECG, and plasma catechloamines and 17-OH corticosteriods in normal man. J. Appl. Physiol. 15:454-458 (as cited in NRC 1996).
Sevel, D., and A. Freedman. 1967. Cerebro-retinal degeneration due to carbon dioxide poisoning. Br. J. Ophthalmol. 51(7):475-482.
Sinclair, R.D., J.M. Clark, and B.E. Welch. 1969. Carbon dioxide tolerance levels for space cabins. Proceedings of the Fifth Annual Conference on Atmospheric Contamination in Confined Spaces, September 16-18, Wright-Patterson Air Force Base, Dayton, OH.
Sinclair, R.D., J.M. Clark, and B.E. Welch. 1971. Comparison of physiological responses of normal man to exercise in air and in acute and chronic hypercapnia. Pp. 409-417 in Underwater Physiology, C.J. Lambertsen, ed. New York: Academic Press.
Staszewska-Barczak, J., and G.J. Dusting. 1981. Importance of circulating angiotensin II for elevation of arterial pressure during acute hypercapnia in anaesthetized dogs. Clin. Exp. Pharmacol. Physiol. 8(3):189-201 (as cited in Eckenhoff and Longnecker 1995).
Storm, W.F., and C.L. Giannetta. 1974. Effects of hypercapnia and bedrest on psychomotor performance. Aerosp. Med. 45(4):431-433.
Sun, M., C. Sun, and Y. Yang. 1996. Effect of low-concentration CO2 on stereoacuity and energy expenditure. Aviat. Space Environ. Med. 67(1):34-39.
Tansey, W.A., J.M. Wilson, and K.E. Schaefer. 1979. Analysis of health data from 10 years of Polaris submarine patrols. Undersea Biomed. Res. 6(Suppl.):S217-S246 (as cited in NRC 1996).
Tashkin, D.P., and D.H. Simmons. 1972. Effect of carbon dioxide breathing on specific airway conductance in normal and asthmatic subjects. Am. Rev. Resp. Dis. 106(5):729-739 (as cited in NRC 1996).
Vandemark, N.L., B.D. Schanbacher, and W.R. Gomes. 1972. Alternations in testes of rats exposed to elevated atmospheric carbon dioxide. J. Reprod. Fertil. 28(3):457-459 (as cited in NRC 1996).
Wamsley, J.R., E.W. Youngling, and W.F. Behm. 1969. High fidelity simulations in the evaluation of environmental stress: Acute CO2 exposure. Aerospace Med. 40:1336-1340 (as cited in NRC 1996).
West, J.B. 1979. Pp. 23, 74 in Respiratory Physiology: The Essentials. Baltimore: Williams and Wilkins.
White, C.S., J.H. Humm, E.D. Armstrong, and N.P.V. Lundgren. 1952. Human tolerance to acute exposure to carbon dioxide. 1. Six Per Cent Carbon Dioxide in Air and in Oxygen. J. Aviat. Med. 23:439-455 (as cited in NRC 1996).
Wilson, A.J., and K.E. Schaefer. 1979. Effect of prolonged exposure to elevated carbon monoxide and carbon dioxide levels on red blood cell parameters during submarine patrols. Undersea Biomed. Res. 6(Suppl.):S49-S56.
Yang, Y., S. Changnian, and M. Sun. 1997. The effect of moderately increased CO2 concentration on perception of coherent motion. Aviat. Space Environ. Med. 68(3):187-191.
Yonezawa, A. 1968. Influence of carbon dioxide inhalation on renal circulation and electrolyte metabolism [in Japanese]. Jpn. Cir. J. 32:119-120 (as cited in NRC 1996).